SCREW FASTENER THEORY & APPLICATIONS - Unbrako's guide
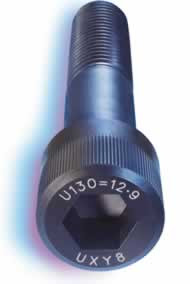
JOINT DESIGN AND FASTENER SELECTION . Joint Length The longer the joint length, the greater the total elongation will occur in the bolt to produce the desired clamp load or preload. In design, if the joint length is increased, the potential loss of preload is decreased. Joint Material If the joint material is relatively stiff compared to the bolt material, it will compress less and therefore provide a less sensitive joint, less sensitive to loss of preload as a result of brinelling, relaxation and even loosening. Thread Stripping Strength Considering the material in which the threads will be tapped or the nut used, there must be sufficient engagement length to carry the load. Ideally, the length of thread engagement should be sufficient to break the fastener in tension. When a nut is used, the wall thickness of the nut as well as its length must be considered. An estimate, a calculation or joint evaluation will be required to determine the tension loads to which the bolt and joint will